Genetic GPS system of animal development explains why limbs grow from torsos and not heads
- Written by Ethan Bier, Professor of Cell and Developmental Biology, University of California San Diego
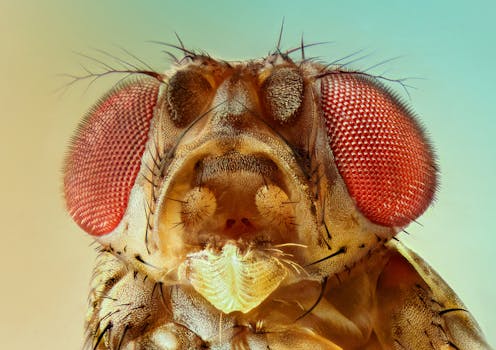
Why do human look like humans, rather than like chimps? Although we share 99% of our DNA[1] with chimps, our faces and bodies look quite different from each other.
While human body shape and appearance have clearly changed during the course of evolution, some of the genes that control the defining characteristics of different species surprisingly have not. As a biologist studying evolution and development[2], I have devoted many years to pondering how genes actually make people and other animals look the way they do.
New research[3] from my lab on how these genes work has shed some light on how genes that have remained unchanged for hundreds of thousands of years can still alter the appearance of different species as they evolve.
Heads versus tails
In biology, a body plan[4] describes how an animal’s body is organized from head to toe – or tail. All animals with bilateral symmetry[5], meaning their left and right sides are mirror images, share similar body plans. For example, the head forms at the anterior end, limbs form in the mid-body, and the tail forms at the posterior end.
Hox genes[8] play an important role in setting up this body plan. This group of genes is a subset of genes involved in anatomical development called homeobox genes[9]. They act like a genetic GPS system, determining what each body segment will turn into during development. They ensure that your limbs grow from your torso instead of from your head by controlling other genes that instruct the formation of specific body parts.
All animals have Hox genes and express them in similar body regions. Furthermore, these genes haven’t changed throughout evolutionary history. How can these genes remain so stable over such vast evolutionary time spans, yet play such pivotal roles in animal development?
Blast from the past
In 1990, molecular biologist William McGinnis[10] and his research team wondered whether the Hox genes from one species might function similarly in another species. After all, these genes are active in similar body regions in animals ranging from fruit flies to humans and mice.
This was a bold idea. As an analogy, consider cars: Most car parts typically are not interchangeable between different makes. The first automobile[11] was only invented around 100 years ago. Compare that to flies and mammals, whose last common ancestor[12] lived over 500 million years ago. It was virtually unthinkable that swapping genes from different species that diverged from each other over such a vast period of time could work.
Nonetheless, McGinnis and his team went ahead with their experiment and inserted mouse or human Hox genes into fruit flies. They then activated the genes in the wrong corresponding areas of the body – for instance, placing the Hox gene that tells a human leg where to develop at the very front of a fruit fly’s head. A misplaced body part would indicate that the mouse or human Hox genes were functioning like the fruit fly’s own genes would have.
Remarkably, both mouse[13] and human[14] Hox genes transformed the fruit fly antennae into legs. This meant that the positional information provided by the human and mouse genes was still recognized in the fly, millions of years later.
How do Hox genes really work?
The next big question, then, was how exactly do these Hox genes determine the identities of different body regions?
There have been two schools of thought on how Hox genes work. The first, called the instructive hypothesis[15], proposes that these shape-controlling genes function as “master” regulatory genes that supply the body instructions on how to develop different body parts.
The second, proposed by McGinnis, hypothesizes that Hox genes instead provide a positional code[16] that marks particular locations in the body. Genes can use these codes to produce specific body structures at those locations. Over the course of evolution, specific body parts come under the control of a specific Hox gene in a way that would best maximize the organism’s survival. This is why flies develop antennae rather than legs on their heads, and humans have collar bones below instead of above their necks.
In a recent study[17] published in the journal Science Advances, a mentee of McGinnis and myself, Ankush Auradkar[18], puts these hypotheses to the test on fruit flies.
Auradkar focused on a fruit fly Hox gene called proboscipedia (pb), which directs the formation of the fly’s mouthparts. He used CRISPR-based genome editing[20] to replace the pb gene from the common laboratory variety of fruit fly, Drosophila melanogaster, or D. mel for short, with its Hawaiian cousin, Drosophila mimica or D. mim. If the instructive hypothesis were correct, D. mel would form D. mim‘s grill-like mouthparts. Conversely, if McGinnis’ hypothesis were correct, D. mel‘s mouthparts should stay the same.
As McGinnis predicted, the flies with the D. mim genes did not develop D. mim’s grill-like features. There was one feature of D. mim’s, however, that did sneak through: Sensory organs called maxillary palps that usually stick out from the face for D. mel were instead aligned parallel to the mouth. This showed that the pb gene provided both a marker for where the mouth should form as well as instructions on how to form it. Though the main outcome favored McGinnis’ theory, both hypotheses were largely correct.
Auradkar also wondered how the pb gene determined the orientation of the maxillary palps. It could have done this by changing the protein it encodes, which carries out the instructions given by the gene. Or it could have changed how it controls other genes, acting like a light switch that determines when and where genes are turned on. Through more testing, he found that this D. mim feature resulted from changing how strongly the pb gene turns on in regions that form the palps, as opposed to changes in the protein itself. This finding highlights once again the remarkable preservation of Hox protein function over evolution – the genetic hardware worked as well in one species as the other.
Auradkar also found that Hox genes engage in an evolutionary tug-of-war with each other. One Hox gene may become more dominant than another and determine what features will ultimately form in a species.
These experiments showed that even subtle changes in how Hox genes interact with each other can have significant consequences for an organism’s body shape.
Hox genes and human health
What do these fly studies mean for people?
First, they provide a window into how the body plans of different species change over the course of evolution. Understanding how Hox genes can manipulate animal development to promote their survival could elucidate why animals look the way they do. Similar mechanisms could explain why humans no longer look like chimps.
Second, these insights may lead to a better understanding of how congenital birth defects[23] arise in people. Changes, or mutations, that disrupt the normal functioning of Hox genes could result in conditions like cleft lip or congenital heart disease. New therapies on the horizon using CRISPR-based genome editing could be used to treat these often debilitating conditions, including muscular dystrophy[24].
[Get the best of The Conversation, every weekend. Sign up for our weekly newsletter[25].]
References
- ^ share 99% of our DNA (www.science.org)
- ^ biologist studying evolution and development (biology.ucsd.edu)
- ^ New research (doi.org)
- ^ body plan (doi.org)
- ^ bilateral symmetry (courses.lumenlearning.com)
- ^ CNX OpenStax/Wikimedia Commons (commons.wikimedia.org)
- ^ CC BY (creativecommons.org)
- ^ Hox genes (doi.org)
- ^ homeobox genes (doi.org)
- ^ William McGinnis (biology.ucsd.edu)
- ^ first automobile (www.loc.gov)
- ^ last common ancestor (doi.org)
- ^ mouse (doi.org)
- ^ human (doi.org)
- ^ instructive hypothesis (doi.org)
- ^ positional code (doi.org)
- ^ recent study (doi.org)
- ^ Ankush Auradkar (scholar.google.com)
- ^ Antonio Quesada Díaz/Wikimedia Commons (commons.wikimedia.org)
- ^ CRISPR-based genome editing (medlineplus.gov)
- ^ Ankush Auradkar (doi.org)
- ^ CC BY-NC-ND (creativecommons.org)
- ^ congenital birth defects (www.who.int)
- ^ muscular dystrophy (doi.org)
- ^ Sign up for our weekly newsletter (theconversation.com)
Authors: Ethan Bier, Professor of Cell and Developmental Biology, University of California San Diego